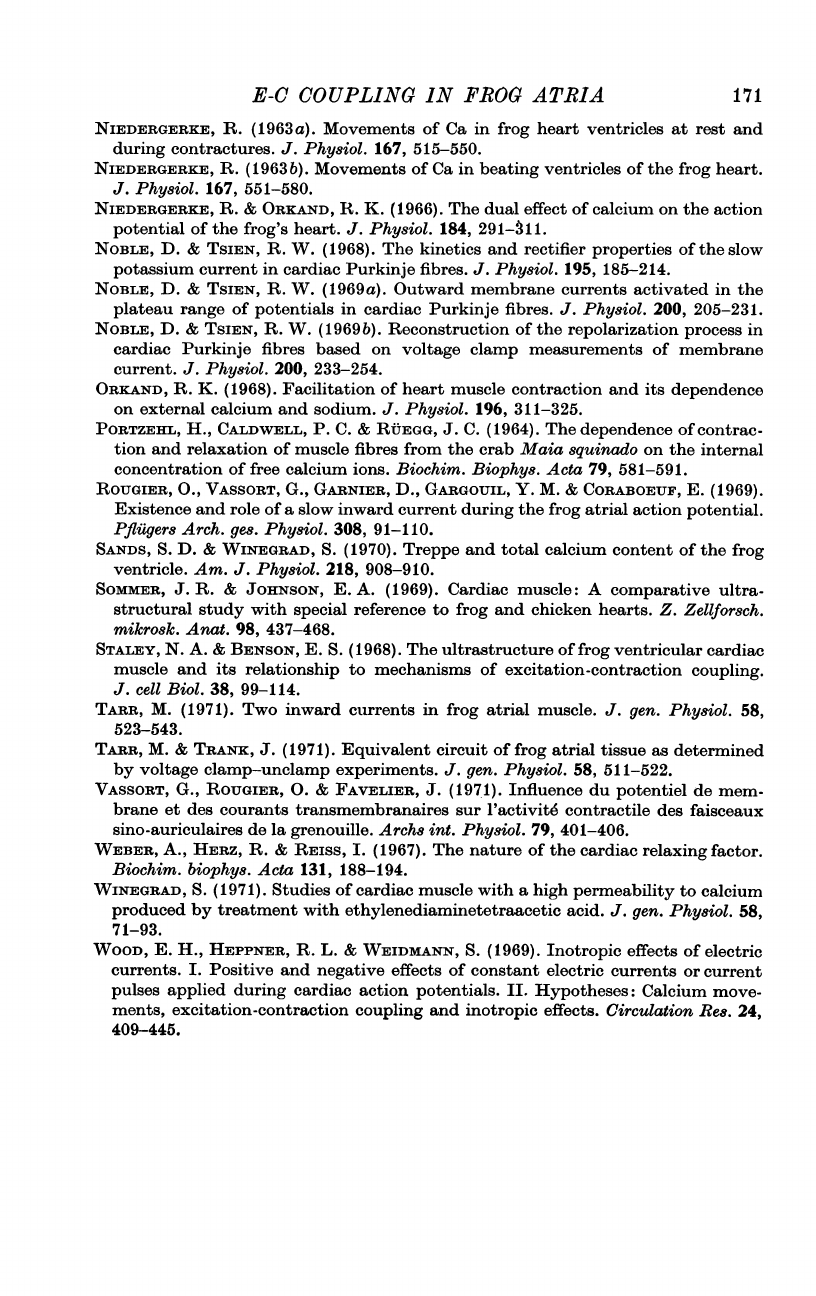
E-G
COUPLING
IN
FROG
ATRIA
171
NIEDERGERKE,
R.
(1963a).
Movements
of
Ca
in
frog
heart
ventricles
at
rest
and
during
contractures.
J.
Physiol.
167,
515-550.
NIEDERGERKE,
R.
(1963b).
Movements
of
Ca
in
beating
ventricles
of
the
frog
heart.
J.
Physiol.
167,
551-580.
NIEDERGERKE,
R.
&
ORKAND,
R.
K.
(1966).
The
dual
effect
of
calcium
on
the
action
potential
of
the
frog's
heart.
J.
Physiol.
184,
291-l11.
NOBLE,
D.
&
TSIEN,
R.
W.
(1968).
The
kinetics
and
rectifier
properties
of
the
slow
potassium
current
in
cardiac
Purkinje
fibres.
J.
Physiol.
195,
185-214.
NOBLE,
D.
&
TSIEN,
R.
W.
(1969a).
Outward
membrane
currents
activated
in
the
plateau
range
of
potentials
in
cardiac
Purkinje
fibres.
J.
Physiol.
200,
205-231.
NOBLE,
D.
&
TsIEN,
R.
W.
(1969b).
Reconstruction
of
the
repolarization
process
in
cardiac
Purkinje
fibres
based
on
voltage
clamp
measurements
of
membrane
current.
J.
Physiol.
200,
233-254.
ORKAND,
R.
K.
(1968).
Facilitation
of
heart
muscle
contraction
and
its
dependence
on
external
calcium
and
sodium.
J.
Physiol.
196,
311-325.
PORTZEHL,
H.,
CALDWELL,
P.
C.
&
RUEGG,
J.
C.
(1964).
The
dependence
of
contrac-
tion
and
relaxation
of
muscle
fibres
from
the
crab
Maia
squinado
on
the
internal
concentration
of
free
calcium
ions.
Biochim.
Biophys.
Acta
79,
581-591.
ROUGIER,
O.,
VASSORT,
G.,
GARNIER,
D.,
GARGOUIL,
Y.
M.
&
CORABOEUF,
E.
(1969).
Existence
and
role
of
a
slow
inward
current
during
the
frog
atrial
action
potential.
Pfluigerm
Arch.
ges.
Physiol.
308,
91-110.
SANDS,
S.
D.
&
WINEGRAD,
S.
(1970).
Treppe
and
total
calcium
content
of
the
frog
ventricle.
Am.
J.
Physiol.
218,
908-910.
SOMMER,
J.
R.
&
JOHNSON,
E.
A.
(1969).
Cardiac
muscle:
A
comparative
ultra-
structural
study
with
special
reference
to
frog
and
chicken
hearts.
Z.
Zellforsch.
mikrosk.
Anat.
98,
437-468.
STALEY,
N.
A.
&
BENSON,
E.
S.
(1968).
The
ultrastructure
of
frog
ventricular
cardiac
muscle
and
its
relationship
to
mechanisms
of
excitation-contraction
coupling.
J.
cell
Biol.
38,
99-114.
TARR,
M.
(1971).
Two
inward
currents
in
frog
atrial
muscle.
J.
yen.
Physiol.
58,
523-543.
TARR,
M.
&
TRANK,
J.
(1971).
Equivalent
circuit
of
frog
atrial
tissue
as
determined
by
voltage
clamp-unclamp
experiments.
J.
yen.
Physiol.
58,
511-522.
VASSORT,
G.,
ROUGIER,
0.
&
FAVELIER,
J.
(1971).
Influence
du
potential
de
mem-
brane
et
des
courants
transmembranaires
sur
l'activit6
contractile
des
faisceaux
sino-auriculaires
de
la
grenouille.
Arch8
int.
Physiol.
79,
401-406.
WEBER,
A.,
HERZ,
R.
&
REISS,
I.
(1967).
The
nature
of
the
cardiac
relaxing
factor.
Biochim.
biophys.
Acta
131,
188-194.
WINEGRAD,
S.
(1971).
Studies
of
cardiac
muscle
with
a
high
permeability
to
calcium
produced
by
treatment
with
ethylenediaminetetraacetic
acid.
J.
yen.
Physiol.
58,
71-93.
WOOD,
E.
H.,
HEPPNER,
R.
L.
&
WEIDMANN,
S.
(1969).
Inotropic
effects
of
electric
currents.
I.
Positive
and
negative
effects
of
constant
electric
currents
or
current
pulses
applied
during
cardiac
action
potentials.
II.
Hypotheses:
Calcium
move-
ments,
excitation-contraction
coupling
and
inotropic
effects.
Circulation
Res.
24,
409-445.